Understanding Thermodynamics: Definitions and Applications
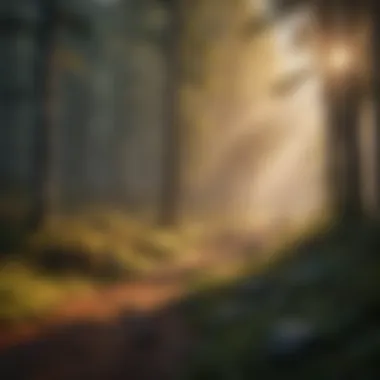
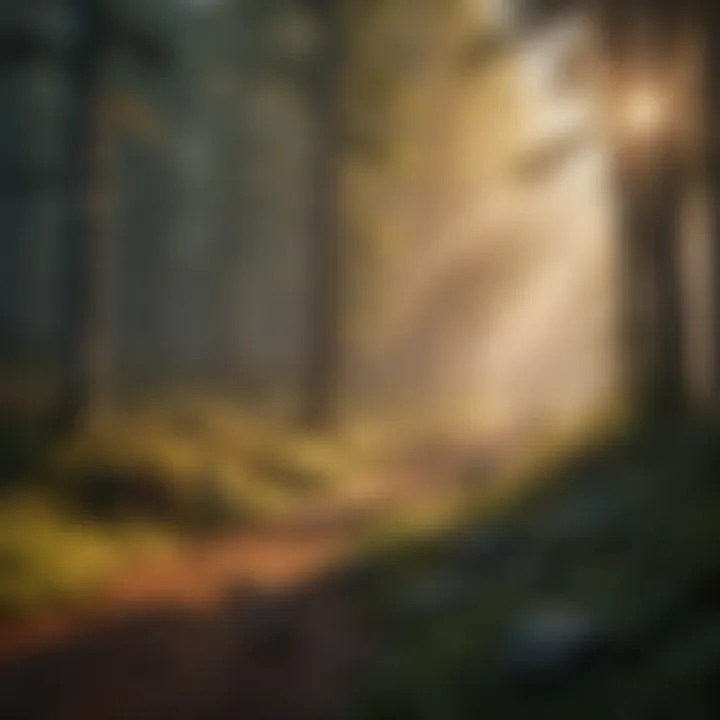
Intro
Thermodynamics is a vast field of study that is crucial for understanding various natural and man-made processes. It serves as a backbone for disciplines such as physics, chemistry, engineering, and even environmental science. The framework of thermodynamics provides insights into energy interactions, transformations, and the inherent laws governing these phenomena. This article will guide readers through the core principles of thermodynamics, emphasizing its real-world applications and implications, particularly in the forestry sector.
The growing concern for sustainable practices in multiple fields has made thermodynamics even more relevant. As we delve deeper into this topic, we will explore the historical aspects, core concepts, and applications of thermodynamics that shape our approach to managing natural resources and addressing environmental challenges.
Understanding Forestry Practices
In the context of thermodynamics, forestry practices play a significant role. These practices relate to how we interact with forests, manage resources, and implement sustainability measures. The dynamics of energy use and conservation within forestry are informed by thermodynamic principles.
Types of Forestry Practices
Forestry encompasses various practices aimed at conserving and managing forest resources effectively. Some notable types include:
- Sustainable Forestry: This practice aims to maintain the forest's biodiversity, productivity, and ecological integrity while meeting the present needs of society.
- Selective Logging: This method involves the careful removal of mature trees, allowing for minimal disturbance to the ecosystem and promoting regeneration.
- Afforestation and Reforestation: These practices focus on planting trees in areas that were not previously forested or replanting trees in degraded or deforested areas.
Historical Context
Understanding the historical context of forestry practices is vital to appreciating modern applications of thermodynamics in this field. Historically, forests were seen primarily as resources for timber and fuel. Over centuries, this led to significant deforestation, prompting a shift in perspective. With advances in ecological understanding, the focus has shifted toward sustainable management, conservation, and restoring forest resources.
Principles of Woodland Stewardship
Woodland stewardship principles encompass a variety of strategies aimed at preserving forest ecosystems. These principles align closely with thermodynamic concepts, whereby energy conservation and efficient resource utilization are fundamental.
Conservation Techniques
Conservation techniques utilized in forestry practices focus on minimizing waste and optimizing resource use. Effective techniques include:
- Utilizing local species to promote biodiversity and resilience.
- Implementing controlled burns to enhance soil fertility and reduce wildfire risks.
- Engaging in proper water management to support healthy forest ecosystems.
Managing Forest Resources
Managing forest resources requires a deep understanding of energy flows and ecological interactions. Using thermodynamic principles informs decision-making regarding resource allocation, forest health, and productivity. This management includes monitoring soil quality, water availability, and species health.
Sustainable Forest Management
Sustainable forest management is an approach that seeks to meet current needs without compromising future generations' ability to meet their own. It is inherently linked to thermodynamics as it considers energy flows and conservation in every practice.
Certification Standards
Various certification standards exist to ensure sustainable practices are being followed in forestry. Notable certifications include Forest Stewardship Council (FSC) and Programme for the Endorsement of Forest Certification (PEFC). These standards offer guidelines that help ensure forests are managed responsibly, taking into account the delicate balance of ecosystems.
Economic Benefits of Sustainability
Adopting sustainable practices can yield considerable economic benefits. Sustainable forestry has the potential to:
- Increase long-term profitability by ensuring resource replenishment.
- Open access to new markets due to certification, linking sustainability with economic growth.
- Enhance ecosystem health, thereby reducing costs associated with restoration efforts in the long run.
"Sustainable practices in forestry not only benefit the environment but also drive economic growth and community resilience."
As we conclude this section, it is evident that thermodynamics lies at the heart of our understanding and management of forestry practices. Its implications on various disciplines evolve as we navigate environmental challenges and seek sustainable solutions.
Prelude to Thermodynamics
Thermodynamics is a fundamental branch of physics that examines the relationships and conversions between heat, work, and energy. Understanding the principles of thermodynamics is crucial, as it touches many facets of our daily lives and drives the design and efficiency of systems across various fields, including forestry, engineering, and environmental science. This introduction aims to highlight the essential elements, benefits, and considerations surrounding thermodynamics to provide a robust framework for further discussion in the subsequent sections of this article.
One significant benefit of studying thermodynamics is its application in energy efficiency. In an era where sustainability and conservation of resources are paramount, thermodynamics provides insights into optimizing energy systems. The principles underpin energy management practices, resource allocation, and environmental modeling, all crucial for effective resource management. By grasping the core aspects of thermodynamics, professionals can better address the complexities of modern energy challenges.
Key elements to consider include:
- The foundational laws of thermodynamics that dictate the behavior of energy systems.
- The historical context that has shaped thermodynamic principles and their applications.
- The relevance of thermodynamics in contemporary issues, including climate change and resource depletion.
Even though thermodynamics can be challenging due to its abstract nature, its real-world applications shed light on many phenomena. Through this exploration, the article aims to connect theoretical concepts to practical implications in various sectors.
"Thermodynamics is not just a set of rules for understanding energy; it is a vital framework for appreciating the processes that govern our world."
Exploring thermodynamics provides valuable insights that can enhance the decision-making processes for forestry professionals and academics alike. The understanding gained from thermodynamics fosters deeper discussions about efficiency, sustainability, and ethics in resource management.
Definition of Thermodynamics
Thermodynamics is a branch of physics that deals with heat, work, and the forms of energy involved in physical and chemical processes. Understanding thermodynamics is crucial, as it lays the scientific foundation for many applications across various fields including engineering, environmental science, and natural resource management. A clear understanding of thermodynamic principles enables professionals to optimize systems, improving efficiency and sustainability.
Fundamental Concepts
Thermodynamics is grounded in several core concepts. These include the notions of system, surroundings, energy, and heat transfer. A system refers to the part of the universe that is under investigation, while the surroundings represent everything outside the system. The transfer of energy can occur in the form of heat and work. It is vital to distinguish between the two as they influence the equilibrium and the state of a system.
The second law of thermodynamics introduces the concept of entropy, which is a measure of disorder or randomness in a system. Higher entropy signifies greater disorder, indicating spontaneous processes tend to increase the overall entropy of the universe. This understanding is fundamental in assessing energy transformations, especially in ecological contexts.
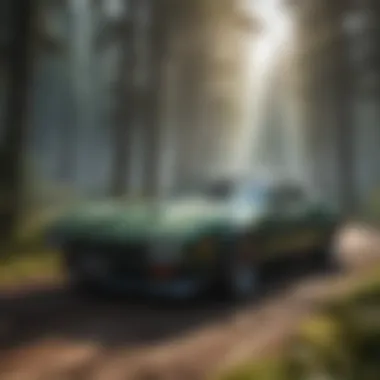
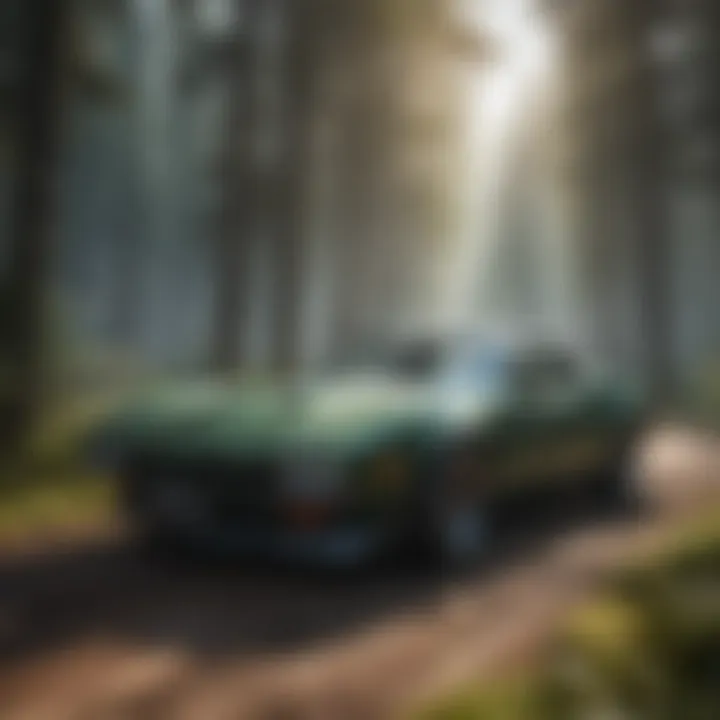
Thermal Energy and Temperature
Thermal energy is a form of energy related to the temperature of a system. It is the total kinetic energy of particles in a substance. As temperature rises, particles move faster, leading to increases in thermal energy. However, temperature itself is a measure of how hot or cold an object is, which is typically measured in degrees Celsius or Kelvin.
The relationship between thermal energy and temperature is pivotal to several phenomena observed in nature and engineering systems. For instance, understanding this relationship is essential in processes like combustion, refrigeration, and energy production. Moreover, in forestry practices, this knowledge guides decisions about species suitability based on climatic conditions, thus impacting biodiversity conservation efforts and resource management.
"Thermodynamics is the science of energy transfer, governing the way heat and work interact in nature."
In synthsis, the definitions and fundamental concepts of thermodynamics inform various disciplines, from improving energy efficiency in industrial processes to making informed decisions in environmental conservation. The principles extend their reach into real-world applications, drawing connections between theory and practical implications.
Core Principles of Thermodynamics
Thermodynamics serves as the backbone for numerous scientific and engineering disciplines. Understanding the core principles of thermodynamics enables professionals to manipulate energy systems effectively. These principles highlight how energy moves and changes within systems and have significant implications for practical applications.
System and Surroundings
In thermodynamics, a system is defined as the part of the universe being studied, while the surroundings comprise everything outside that system. This distinction allows scientists to isolate variables and analyze energy interactions effectively.
One vital consideration in thermodynamics is the various types of systems:
- Closed systems can exchange energy but not matter with their surroundings.
- Open systems exchange both energy and matter.
- Isolated systems do not exchange either with their surroundings.
Understanding how systems interact with their surroundings is critical when designing energy-efficient models, particularly in fields like forestry and environmental science.
Dealing with these boundaries helps capture the dynamics of energy transfer, revealing insights into energy conservation principles that are foundational within the discipline.
Equilibrium and State Functions
Equilibrium refers to the state in which all driving forces in a thermodynamic system are balanced. In equilibrium, there are no net changes in the system's properties over time. Achieving thermal, mechanical, and chemical equilibrium is essential for steady-state operations.
State functions are properties that depend only on the current state of the system, not on how it reached that state. Key state functions include:
- Internal Energy (U)
- Enthalpy (H)
- Entropy (S)
These state functions help quantify energy exchanges in thermodynamic processes. They simplify the analysis of cycles, such as the Carnot cycle and Rankine cycle, making it easier for engineers and scientists to assess performance.
"The mastery of state functions enables precise calculations, facilitating groundbreaking advancements in energy systems."
By relying on these core principles, professionals can evaluate system efficiency and stability, leading to better design practices.
Understanding the core principles of thermodynamics is essential for addressing complex energy challenges. These principles link theoretical knowledge with practical implementations, guiding innovations in sustainable practices and resource management.
Laws of Thermodynamics
The laws of thermodynamics serve as the foundation of thermal physics, defining how energy interacts within systems. Understanding these laws is crucial in providing insights into energy transfer and conversion processes, important not just in the realm of physics but also across engineering and environmental science. In this discussion, we will look at each law individually, emphasizing its significance and implications in various fields of study.
Zeroth Law of Thermodynamics
The Zeroth Law of Thermodynamics establishes a fundamental principle of temperature measurement. It states that if two systems are in thermal equilibrium with a third system, then they are in thermal equilibrium with each other. This concept underpins the very notion of temperature. It allows for the use of thermometers and other temperature-measuring devices, making it vital in scientific and engineering applications. The simplicity of this law belies its importance, contributing to our understanding of how energy is transferred and conserved in thermal processes.
"The Zeroth Law provides the basis for temperature measures that enable comparison of thermal states across systems."
First Law of Thermodynamics
The First Law of Thermodynamics, often referred to as the Law of Energy Conservation, posits that energy cannot be created or destroyed, only transformed from one form to another. In practical terms, this means that the total energy of an isolated system remains constant. This principle is fundamental to understanding energy flow in mechanical systems, biological organisms, and even planetary processes. It highlights the importance of energy efficiency, where systems must maximize energy output while minimizing waste.
Second Law of Thermodynamics
The Second Law of Thermodynamics introduces the concept of entropy, stating that in any energy exchange, if no energy enters or leaves the system, the potential energy of the state will always be less than that of the initial state. It emphasizes that natural processes tend to move towards a state of greater disorder. This law has profound implications in various fields, including engineering, environmental science, and thermodynamics. It informs us about the efficiency of engines and refrigeration systems while also addressing broader issues like heat death in the universe.
Third Law of Thermodynamics
The Third Law of Thermodynamics states that as the temperature of a perfect crystal approaches absolute zero, the entropy approaches a constant minimum. This principle provides insight into the behavior of materials at very low temperatures and helps to establish the absolute scale of entropy. Its applications are significant in fields such as cryogenics and low-temperature physics. The Third Law assures that itβs physically impossible to reach absolute zero, influencing how researchers understand energy and matter at extreme conditions.
In summary, the laws of thermodynamics are essential not only for theoretical physics but also for practical applications in various scientific disciplines. Each law builds on the previous, collectively contributing to a deeper understanding of how energy behaves in our universe.
Applications of Thermodynamics
The applications of thermodynamics extend into various fields, encompassing engineering, environmental science, and resource management. Understanding these applications is crucial, as thermodynamic principles guide the design and operation of systems that influence our daily lives and the environment. This section highlights the relevance of thermodynamics across diverse domains and emphasizes its significance in tackling contemporary challenges.
Thermodynamics in Engineering
In engineering, thermodynamics is foundational. It plays a vital role in designing systems like engines, refrigerators, and power plants. Engineers apply thermodynamic concepts to analyze energy conversions, optimize performance, and improve efficiency. For instance, the First Law of Thermodynamics is often employed in heat engines to evaluate work output versus energy input. By understanding the heat transfer and energy distributions, engineers can develop more efficient technologies that consume less fuel and emit fewer pollutants.
Key Engineering Applications:
- Power Generation: Thermodynamic cycles such as the Rankine and Brayton cycles are essential for power plant operations. They allow engineers to maximize electricity generation while minimizing energy losses.
- Refrigeration and Air Conditioning: Thermodynamics is at the core of designing cooling systems. The Second Law ensures that heat moves from a cooler area to a warmer one, guiding design in refrigeration units.
- Material Processing: The principles help in controlling temperatures and processes in the fabrication of materials, ensuring quality and performance.
Role in Environmental Science
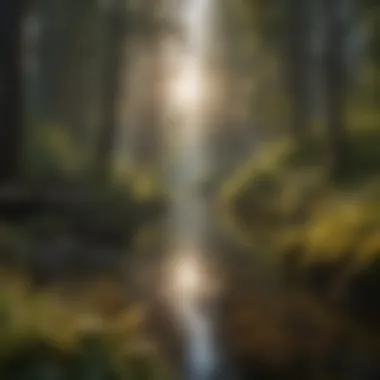
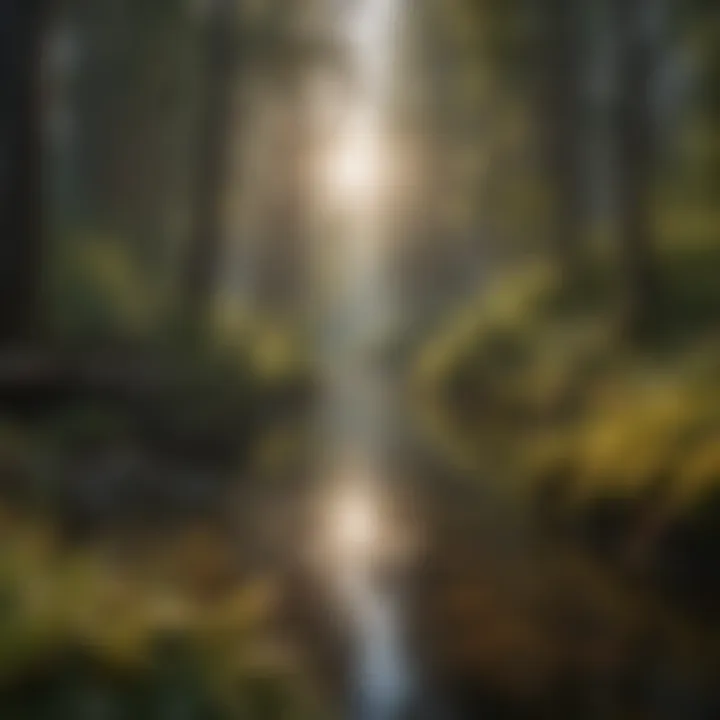
Thermodynamics significantly impacts environmental science. Understanding energy flows within ecological systems is essential for developing sustainable practices and conservation strategies. The laws of thermodynamics help explain energy transfer in natural environments, promoting effective management of resources.
Environmental Science Applications:
- Climate Modeling: Thermodynamic principles are crucial for understanding atmospheric dynamics and energy balances, providing insights on climate change.
- Pollution Control: Engineers use thermodynamic models to assess the efficacy of pollution control technologies, such as waste incineration and gas scrubbing, ensuring the reduction of harmful emissions.
- Renewable Energy Systems: Here, thermodynamics facilitates the design and optimization of solar collectors and wind turbines, maximizing energy harnessing and reducing dependence on fossil fuels.
Impact on Forestry Practices
In forestry, thermodynamics aids in understanding heat dynamics within wood, tree growth processes, and forest ecology. This understanding is vital for developing management strategies that enhance forest productivity and sustainability.
Forestry Applications:
- Growth Modeling: Thermodynamic principles can predict how temperature and moisture affect tree growth, which is exciting for forestry management.
- Carbon Sequestration: Understanding energy transformations in forest ecosystems helps quantify the amount of carbon dioxide captured by trees, informing climate mitigation strategies.
- Forest Fire Management: Knowledge of thermodynamic properties of materials can predict fire behavior, facilitating better strategies to manage and contain wildfires.
"Thermodynamics influences every aspect of energy transfer, making it a key component of engineering design, environmental analysis, and resource management."
By illustrating the applications of thermodynamics in these varied fields, we see how they inform our understanding and management of critical systems. The comprehension of these principles not only advances technology but also supports the sustainable development objectives pertinent to our society.
Thermodynamic Cycles
Thermodynamic cycles are fundamental processes in which a working substance undergoes a series of state changes, ultimately returning to its initial state. This concept is critical in the study of thermodynamics, especially for understanding how energy is converted from one form to another. The significance of thermodynamic cycles lies in their applications in various engineering systems such as engines, refrigerators, and heat pumps. They illustrate efficiency and performance metrics which are vital for optimizing these systems.
Understanding thermodynamic cycles can significantly enhance energy utilization in numerous applications. They allow for the analysis of how much useful work can be extracted from a system compared to the amount of energy supplied. Different cycles also address the challenges posed by energy loss due to inefficiencies in large systems. Therefore, grasping the principles of these cycles is essential for forestry professionals and academics focused on advancing sustainable technology and resource management.
Thermodynamic cycles exemplify how systems can transform energy and return to their original states, making them a core concept in engineering applications.
Carnot Cycle
The Carnot cycle is an idealized thermodynamic cycle that serves as a standard for measuring the efficiency of real-world engines. It consists of four reversible processes: two isothermal and two adiabatic. In the isothermal expansion step, the working substance absorbs heat from a hot reservoir. Next, it undergoes adiabatic expansion, which allows it to do work without heat transfer. Then, during isothermal compression, the substance releases heat to a cold reservoir. Finally, adiabatic compression completes the cycle by increasing the substance's pressure and temperature.
This cycle establishes the maximum possible efficiency of any heat engine operating between two heat reservoirs.
Rankine Cycle
The Rankine cycle is widely used in power generation, particularly in steam engines and thermal power plants. It consists of four stages: pumping the liquid phase, heating it in a boiler, expansion through a turbine, and condensation back to liquid. The efficiency of the Rankine cycle depends significantly on factors such as the temperature of the heat source and the pressure of the steam. Improvements to this cycle can lead to substantial energy savings and reduced waste heat. In practical applications, the Rankine cycle can be modified to enhance performance through the use of regenerative heat exchangers or superheating, thus capturing more energy from the fuel consumed.
Otto Cycle
The Otto cycle is the thermodynamic cycle that outlines the operation of gasoline engines. It has two major phases: the compression and the power phases, each containing two strokes. Initially, the air-fuel mixture is compressed, then ignited, leading to a rapid expansion that generates power. This cycle is characterized by its reliance on spark ignition to initiate combustion, differing from heat engines that operate under compression ignition. The efficiency of the Otto cycle varies depending on the compression ratio and the design of the engine. Enhancements to the Otto cycle through optimized engine designs, fuel types, and operating conditions can lead to better fuel efficiency and lower emissions, relevant factors in current environmental considerations.
Thermodynamic Properties
Thermodynamic properties are crucial for understanding the behavior of systems in thermodynamics. Each property provides insights into how systems interact under varying conditions. Focusing on elements like internal energy, enthalpy, and entropy is vital. These properties not only define how energy is transferred but also influence processes across many disciplines including engineering, environmental science, and forestry.
Understanding these properties is essential to optimize energy systems, manage resources, and develop sustainable practices. By grasping how these thermodynamic properties function, professionals can make informed decisions on energy conservation and resource allocation in their respective fields.
Internal Energy
Internal energy is the total energy contained within a system. This includes both kinetic and potential energy of particles. It is important for determining the state of a system. When energy is added to a system, its internal energy increases, and conversely, it decreases when energy is removed.
The formula for calculating changes in internal energy is:
[ \Delta U = Q - W ]
Where:
- ( \Delta U ) is the change in internal energy.
- ( Q ) is the heat added to the system.
- ( W ) is the work done by the system.
As such, internal energy plays an ignition role in many thermodynamic processes. Knowing how to manipulate this property can assist in designing more efficient systems, especially in engineering applications that require precise calculations of energy gains and losses.
Enthalpy
Enthalpy is a measure of the total energy of a thermodynamic system, including internal energy and the product of pressure and volume. It is often symbolized as ( H ) and is essential for processes occurring at constant pressure, such as chemical reactions in open systems. The relationship for enthalpy can be expressed as:
[ H = U + PV ]
Where:
- ( H ) is the enthalpy.
- ( U ) is the internal energy.
- ( P ) is the pressure.
- ( V ) is the volume.
Enthalpy is especially significant in fields like environmental science and forestry where heat exchanges are considerable. It helps in analyzing energy transfer during phase changes and reactions, thus influencing strategies for energy efficiency and resource management.
Entropy
Entropy is a measure of the disorder or randomness of a system. It is fundamentally linked to the second law of thermodynamics, which states that the total entropy of an isolated system can never decrease over time. Therefore, understanding entropy is essential for grasping how energy is dispersed in any given process.
Entropy is represented as ( S ) and is calculated using the equation:
[ S = k , ln , racWT ]
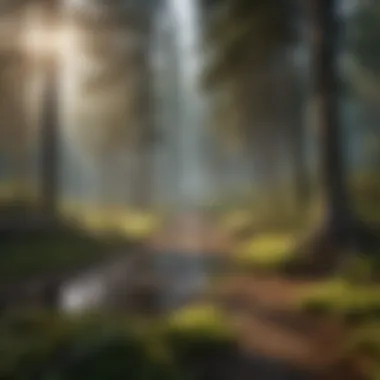
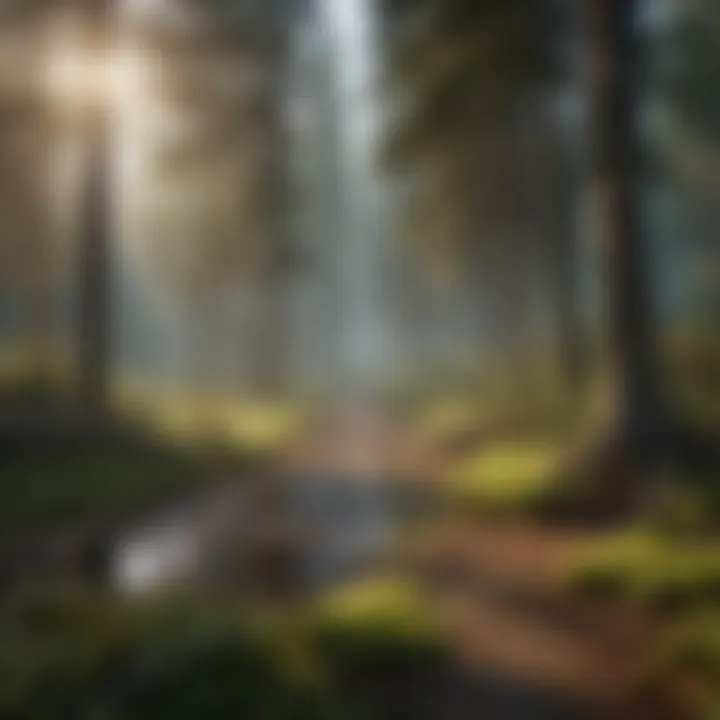
Where:
- ( S ) is the entropy.
- ( k ) is the Boltzmann constant.
- ( W ) is the number of microstates.
- ( T ) is the temperature in Kelvin.
In practical applications, entropy helps to assess the efficiency of energy transformations. High entropy indicates a high level of disorder and lost usable energy. Understanding this concept can aid forestry professionals in making decisions regarding resource utilization and sustainability practices.
"Understanding thermodynamic properties enables the design of more effective systems, leading to substantial gains in energy efficiency."
Thermodynamic properties, namely internal energy, enthalpy, and entropy, form the foundation of thermodynamics. Grasping these concepts is essential for addressing challenges in various fields, enhancing energy efficiency, and promoting sustainable practices.
Thermodynamics and Sustainability
Thermodynamics plays a crucial role in addressing sustainability challenges faced by society today. As we strive to balance economic growth with environmental protection, thermodynamic principles help in understanding energy transformations and efficiency improvements. The laws of thermodynamics provide a framework for evaluating energy systems and their impacts on natural resources. In this context, understanding thermodynamics is essential for developing sustainable practices in energy production, resource management, and waste reduction.
Thermodynamics assists in identifying pathways to reduce energy consumption, leading to greater energy efficiency. By optimizing processes, we can decrease the overall energy input required for various applications. This not only benefits organizations economically but also mitigates environmental impacts. The practical implications of these thermodynamic concepts extend to various sectors, including industry, forestry, and agriculture, reinforcing the importance of integrating sustainability into operations.
The role of thermodynamics in sustainability includes:
- Enhancing energy conversion processes: By studying thermodynamic cycles, we can improve energy conversion technologies, such as heat pumps and engines.
- Fostering renewable energy adoption: Thermodynamic analyses guide the development of solar, wind, and geothermal systems, making them more efficient and practical.
- Minimizing waste: Applying thermodynamic principles leads to better waste management strategies by optimizing resource use and recycling processes.
"Sustainability is not merely the responsibility of a single sector. It requires synergy between technology, resource management, and thermodynamic efficiency."
Energy Efficiency
Energy efficiency is a fundamental aspect of sustainability, which can be greatly enhanced through thermodynamic understanding. In practical terms, energy efficiency involves using less energy to achieve the same output, often achieved by improving processes, equipment, and systems. In industries, this means re-evaluating production methods to decrease energy waste. The savings can be substantial when using thermodynamic principles to analyze energy flow and identify inefficiencies.
For instance, focusing on the efficiency of thermal systems can lead to:
- Improved building HVAC systems: Designing better heating, ventilation, and air conditioning systems can significantly reduce energy consumption in buildings.
- Enhanced industrial processes: Industries employing thermodynamic analysis can reconfigure equipment to optimize energy use, decreasing cost and emissions.
Ultimately, the integration of energy efficiency strategies underpinned by thermodynamics supports corporate social responsibility goals and regulatory compliance.
Resource Management
Resource management is another pivotal aspect influenced by thermodynamic principles. At its core, effective resource management seeks to utilize natural resources sustainably, ensuring that current needs do not compromise future generations. Thermodynamics provides the necessary tools to analyze resource flows and consumption patterns, allowing for more informed decision-making.
In forestry, for example, thermodynamic analyses can help assess the sustainability of resource extraction processes, ensuring that timber harvests are balanced with ecosystem health. Likewise, in agriculture, understanding the energy inputs for different farming practices can lead to more sustainable land use strategies.
Key components of effective resource management include:
- Energy-intensive processes evaluation: Fine-tuning agricultural and forestry operations to minimize energy inputs while maximizing outputs.
- Waste reduction strategies: Implementing thermodynamic assessments to minimize waste throughout the supply chain, from production to consumption.
Challenges and Future Directions
As we navigate through the complexities of thermodynamics, it is essential to consider the challenges that lie ahead. These challenges are not merely obstacles; rather, they represent opportunities for innovation and improvement within our understanding and application of thermodynamic principles. The energy landscape is rapidly evolving. We must adapt to new scientific findings and emerging technologies. This section explores the key aspects of technological advancements and research opportunities that shape the future of thermodynamics.
Technological Advancements
Technological advancements are reshaping how we approach thermodynamic applications. Innovations in materials science, for instance, have the potential to create more efficient energy conversion processes. Advanced thermal insulation materials can significantly reduce energy loss in various applications. This progress could lead to substantial improvements in building design and energy management systems.
Moreover, the integration of artificial intelligence into thermodynamic modeling optimizes energy systems. By simulating various scenarios, AI can help predict outcomes more accurately. These predictions can inform decisions about resource allocation, leading to improved sustainability.
- Improved Energy Efficiency: New technologies are capable of processing energy more efficiently, reducing waste and costs.
- Alternative Energy Sources: Innovations are emerging in harnessing solar and wind energy, aligning with thermodynamic laws to maximize output.
This convergence of emerging technologies fosters a more sustainable future. However, to fully embrace these advancements, professionals must keep detailed records and continuously monitor performance metrics.
Research Opportunities
Research opportunities in thermodynamics are abundant and critical for future progress. There is a pressing need to investigate renewable energy systems. Researchers can explore how thermodynamic principles apply to biofuels, geothermal energy, and hydrogen production. Each of these areas presents unique challenges and a plethora of potential innovations.
In addition, understanding the implications of thermodynamics on climate change is vital. Scholars can delve into studying the thermodynamic cycles of natural ecosystems. This would contribute to developing sustainable forestry practices and improving resource management methodologies.
"Addressing the challenges of thermodynamics requires a collaborative approach among scientists, engineers, and policy makers."
Some vital areas of research include:
- Carbon Capture Technologies: Understanding how thermodynamic cycles can support carbon sequestration processes.
- Energy Storage Solutions: Exploring the efficiencies of thermal batteries and other storage systems to improve grid stability.
Efforts in these domains will not only broaden the academic understanding of thermodynamics but also enhance its practical applications. As advancements in technology and research develop, professionals in forestry and environmental science will benefit from applying these principles to achieve long-term sustainability.
Finale
The conclusion serves a significant role in encapsulating the essence of thermodynamics discussed in this article. It reinforces the key principles, applications, and implications of thermodynamics in various fields. Understanding how thermodynamics influences systems enables professionals to make more informed decisions in engineering, environmental science, and resource management.
Through summarizing the main components of thermodynamic laws and properties, readers can appreciate their practical applications and relevance. Additionally, the conclusion highlights the importance of sustainable practices in thermodynamics. As global challenges, like climate change and energy efficiency, gain prominence, the principles of thermodynamics will guide effective solutions.
Summary of Key Takeaways
- Thermodynamics as a Foundation: This field forms the backbone of many scientific and engineering disciplines.
- Four Laws: The laws of thermodynamics explain energy transfer processes and their implications.
- Applications: Practical uses span engineering, environmental science, forestry, and more, showcasing versatility.
- Sustainability: Understanding thermodynamics directly contributes to enhanced resource management and sustainable practices.
Final Thoughts on Thermodynamics
Thermodynamics remains a cornerstone of science and engineering, influencing numerous applications and decision-making. A profound understanding of its principles allows professionals to tackle complex challenges in their fields effectively. It is essential for both academic and practical purposes, as it lays the groundwork for future innovations.
By integrating thermodynamic knowledge into everyday practices, individuals and organizations can foster a more sustainable future while maximizing efficiency. Thermodynamics is not just about equations and laws; it is about understanding the universe and making choices that lead to a better world.